The Big Questions is a miniseries that explores how technology is helping to investigate some of the deepest and most thought-provoking questions of our existence.
Why isn’t the universe boring? It could be. The number of subatomic particles in the universe is about 1080, a 1 with 80 zeros after it. If these particles were scattered randomly, the universe would just be a dull wasteland of sameness, a thin void with no structure much larger than an atom for billions of light years in any direction. Instead, we have a universe filled with stars and planets, canyons and waterfalls, pine trees and people. There is an exuberant abundance in nature. But why are all these things here?
Cosmologists have pieced together an answer to this question over the past half century, using a variety of increasingly complex experiments and observational instruments. But, as is almost always the case in science, this answer is incomplete. Now, with stunning new sensitivity experiments, physicists hope to detect a never-before-seen event that could explain one of the great remaining mysteries of this story: why there was matter to form complicated things in the first place.
The interest of the world around us is even more intriguing when looking at the universe on larger scales. You find structured clusters for a while. Stars form galaxies, galaxies form clusters of galaxies, and these clusters form superclusters, filaments, and walls around great cosmic voids nearly empty of matter.
But when you zoom out even further, looking at parts of the universe more than 300 million light-years wide, all this structure disappears. After this point, the light from all the stars in the cosmos merges into an indistinct blur, and the universe indeed appears very similar in all directions, with no notable features or differences. Cosmologists call this the “end of greatness.”
This boring cosmic landscape exists because the universe really was once boring. Right after the Big Bang, and for hundreds of thousands of years after that, it was relentlessly dull. All that existed was a thick, incandescent mist of particles, which stretched for trillions and trillions of kilometers and filled every point in the universe almost uniformly, with minuscule differences in the density of matter between one point and another.
But as the universe expanded and cooled, gravity amplified these small differences. Slowly, over the next millions and billions of years, places in the universe with a little more material attracted even more material. And that’s where we came from – the profusion of things in today’s universe ended up emerging as more and more material accumulated, transforming those slightly dense regions into radically complicated places, filled with enough matter to form stars, galaxies and us. On the broadest scales, boredom still reigns, as it has since the beginning of time. But down here, in the dirt, there is great variety.
This story still has some gaps. Firstly, it is not clear where the matter came from. Particle physics demands that anything that creates matter must also create an equal amount of antimatter, carefully maintaining the balance between the two. Every type of matter particle has an antimatter twin that behaves like matter in almost every way. But when a matter particle comes into contact with its antimatter counterpart, they annihilate each other, disappearing and leaving behind nothing but radiation.
This is exactly what happened right after the Big Bang. Matter and antimatter annihilated each other, leaving our universe ablaze with radiation – and a small amount of remaining matter, which had slightly exceeded the amount of antimatter at the start. This small mismatch made the difference between the universe we have today and an eternity of boredom, and we don’t know why that happened. “Somehow, there was this little imbalance and it turned into everything, namely us. I really care about us,” says Lindley Winslow, an experimental particle physicist at MIT. “We have many questions about the universe and how it evolved. But this is a very basic, kindergarten-type question: why are we here?”
Caught in the act To answer this question, Winslow and other physicists around the world have constructed several experiments to capture nature in the act of violating the balance between matter and antimatter. They expect to see this violation in the form of neutrinoless double beta decay, a type of radioactive decay. At the moment, this process is theoretical – it may not happen at all. But if it occurs, it would provide a possible explanation for the imbalance between matter and antimatter in the early universe.
This explanation would be based on neutrinos, the strange ghostly balls of particle physics. These light specters buzz around the universe, barely interacting with anything. Trillions of neutrinos constantly pass through every square inch of your body and throughout planet Earth, ignoring it as completely as they ignore our planet’s iron core. To reliably stop just one neutrino would require a lead slab a light year thick.
E os neutrinos podem realizar um truque ainda mais bizarro. O neutrino e seu parceiro de antimatéria poderiam ser um e o mesmo, tornando-o diferente de todas as outras formas conhecidas de matéria e capaz de se aniquilar. “Se observarmos [o decaimento duplo beta sem neutrino], isso provaria que o neutrino é sua própria antipartícula”, diz Winslow. “Isso também nos forneceria um processo que produz mais matéria do que antimatéria.”
And neutrinos can perform an even more bizarre trick. The neutrino and its antimatter partner could be one and the same, making it different from all other known forms of matter and capable of annihilating each other. “If we look at [neutrinoless double beta decay], that would prove that the neutrino is its own antiparticle,” says Winslow. “It would also provide us with a process that produces more matter than antimatter.”
This process begins in the heart of the atom. When some unstable atomic nuclei decay, they emit an electron along with an antineutrino to counterbalance: a matter particle and an antimatter particle. This is a very common type of radioactive decay, known for historical reasons as beta decay. Much less common is double beta decay, when an atomic nucleus emits two electrons at once, along with two antineutrinos to balance them.
Double beta decay is “one of the longest processes we’ve ever measured,” says Winslow. To see a single atom undergo double beta decay, she continues, we would normally have to wait a billion times longer than the current age of the universe. But if the neutrino is its own antiparticle, there is the possibility of something even rarer than that: a double beta decay in which the two neutrinos immediately annihilate each other, leaving just the two electrons with no antimatter to counterbalance them. This is neutrinoless double beta decay.
Detecting such a rare process would be difficult – but not impossible, thanks to the astronomical number of atoms in ordinary-sized objects. There are almost a trillion trillion atoms in a few grams of material. “So if you stack a bunch of things together, you have the possibility of seeing something that happens on timelines even longer than the age of the universe,” says Winslow.
This is the approach taken by the Cryogenic Underground Observatory for Rare Events (CUORE, its Italian acronym means “heart”), a detector under a mountain in Italy that is awaiting evidence of neutrinoless double beta decay. A particular isotope of tellurium is one of the nuclei susceptible to double beta decay. CUORE observes this phenomenon in an array of 988 five-centimeter-wide cubic crystals of tellurium dioxide, each connected to a highly sensitive thermometer. The combined energy of the two electrons emitted in neutrinoless double beta decay is always the same, so if the decay occurs anywhere within one of these crystals, that specific amount of energy will be deposited in the crystal as heat, increasing its temperature by a ten thousandth of a degree Celsius.
But such a small signal is difficult to see compared to all the other things that could change a crystal’s temperature. This is why CUORE is under a mountain – most of the rock above it protects it from almost all cosmic rays. And that’s also why CUORE needs to be kept cold, just a few thousandths of a degree above absolute zero—it “wins the award for coldest cubic meter in the known universe,” says Winslow. The sensors are so exquisitely delicate that they can even pick up the vibrations of waves crashing onto the beach 60 kilometers awayg amorew.
CUORE is not alone. There are other experiments that look for neutrinoless double beta decay, including KamLAND-Zen, an experiment – also under a mountain – in Japan that uses gaseous xenon in place of tellurium crystals. But none of the experiments looking for the decay have seen it yet, despite years of waiting. There are plans to upgrade the sensors on CUORE and increase the number of crystals being used; There are also plans to increase the size and sensitivity of KamLAND-Zen. But the future of these experiments is uncertain.
“In principle, we could do bigger and better experiments,” says Reina Maruyama, a Yale physicist who is also part of the CUORE collaboration. “You could make 10 of what we have. So I think it’s just a question of how many resources humanity wants to put into this experiment.” Winslow calculates that full research would require two more rounds of improvements to existing experiments. If they are made and come to nothing, she says, “then we will have virtually eliminated the possibility of the neutrino being its own antiparticle.”
If that happens, it will be the end of a promising theory, but not the end of the research. Physicists have many other ideas about how matter and antimatter could have become unbalanced. But it’s hard to find evidence for these ideas. Some could be confirmed if the Large Hadron Collider, the world’s largest particle collider, finds something unexpected in the coming years; other theories rely on sensitive searches for dark matter, a hypothetical, invisible substance, strongly suggested by decades of evidence, that is believed to make up more than 80% of the matter in the universe. And some theories charge a high price to explain the imbalance: they suggest that protons, one of the main components of atomic nuclei, are unstable. These theories say that proton decay takes even longer than neutrinoless double beta decay, on average about a trillion trillion times longer than the current age of the universe.
Super-Kamiokande (also known as “Super-K”) in Japan is the largest proton decay observation experiment, using an underground vat of 50,220 metric tons of ultrapure water surrounded by 13,031 light sensors. At the edge of knowledge, Super-K waits for a faint flash in the darkness. He has yet to capture a proton in action.
But whatever the cause of the imbalance between matter and antimatter in the early universe, there is one thing physicists are certain of: eventually, the show will end. Over time, all interesting structures will disappear as the universe’s matter and energy are scattered more and more randomly. A few eons from now, this will once again lead to a completely featureless void – and this time, it will be much less dense and much more uniform than the primordial mist. This state, known as heat death, will likely be the final fate of the universe, a myriad of quadrillion years in the future.
So we are lucky – we live in a time when the universe is filled with complexity and beauty, even if we don’t fully understand why.
( source: MIT Technology Review )
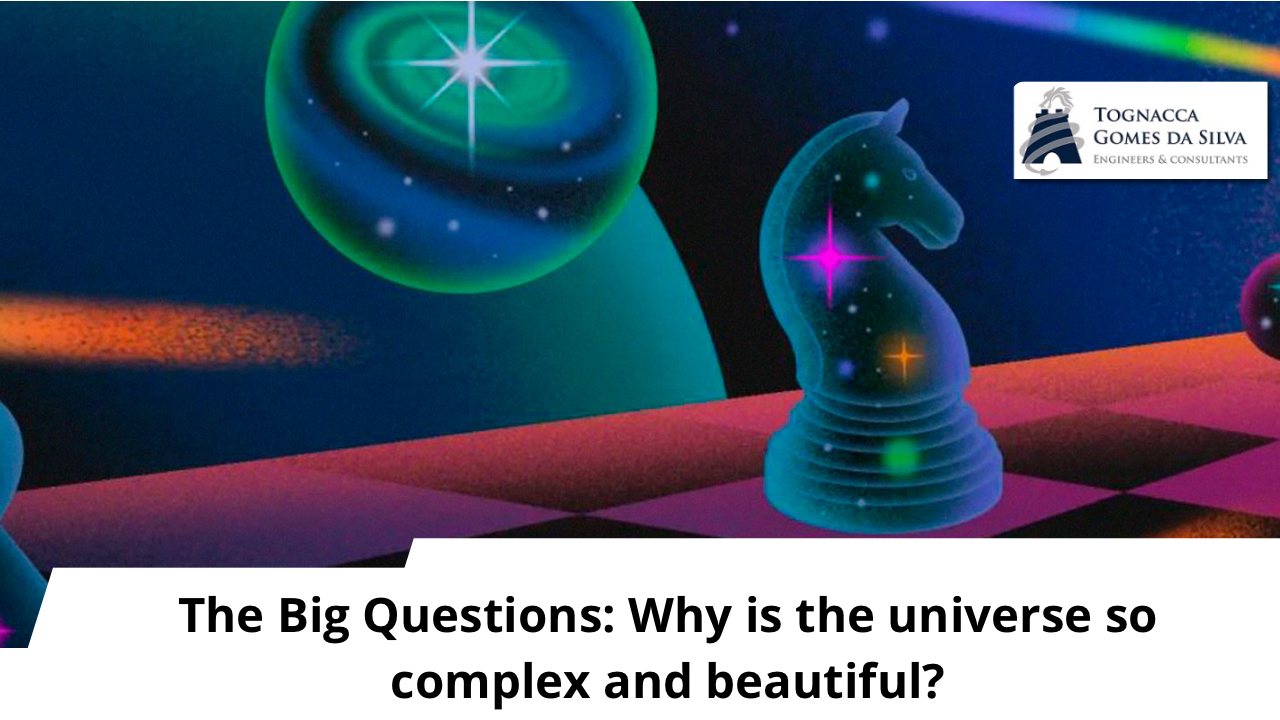